1 Introduction
Peri-urban areas are defined as intersection zones between urban and rural areas where agricultural, industrial, and urban activities converge (Huang et al. 2018). They are important for the provision of ecosystems services such as food production, mitigation of environmental changes, and acting as buffer zones that protect fauna and neighboring ecosystems from human intervention (Livesley et al. 2016; Huang et al. 2018; Kibblewhite 2018). However, rapid expansion of peri-urban areas (attributed to fast population growth and migration; Blanes, 2006; Imbrenda et al., 2021) coupled with inadequate territorial planning, deficient waste management, the use of wastewaters for irrigation and excessive use of agrochemicals may led to environmental degradation (Zhao et al. 2019; Imbrenda et al. 2021). For example, soils of peri-urban areas have been reported to be enriched with potentially toxic elements (PTEs) which include essential elements for organisms that may be toxic at high concentrations (e.g. copper, zinc, manganese; Huang et al. 2018; Kibblewhite 2018; Keshavarzi et al. 2019), or elements that do not have a biological role and are toxic even at low concentrations (e.g. lead, cadmium, chromium, mercury; Wada 2004; Antoniadis et al. 2019; Kaur et al. 2021). Despite that PTEs are naturally present in the environment, high concentrations in the soil may affect soil processes (i.e., organic matter decomposition, nitrification) or enter the trophic chain via plant uptake, affect food quality and be hazardous for humans and animals (Fang et al. 2011; Mahurpawar 2015; Huang et al. 2018; Kibblewhite 2018; Keshavarzi et al. 2019; Kaur et al. 2021).
Accumulation of PTEs in agricultural soils of peri-urban areas has been attributed to the proximity of agricultural fields to urbanized and industrial areas. PTEs originated from domestic and industrial activities may be transported by water to neighboring canal, rivers, or lakes, and accumulate in the sediments (Hakanson 1980; Zhang et al. 2015). Sediments would thus act as sink and source of PTEs and play an important role in determining their mobilization (Zhang et al. 2015). Water or sediments containing PTEs may ultimately contribute to PTEs accumulation in soils. Accordingly, several studies have reported PTEs present in water and sediments may lead to contamination of agricultural soils with the potential to be hazardous in the long-term (Miller et al. 2004; Mapanda et al. 2005). Moreover, given that PTEs accumulation in soils may be a slow process (depending on the PTEs loads; Zhang et al. 2015), evaluation of the PTEs present in sediments could indicate which elements are likely to accumulate in soils overtime. Evaluation of PTEs present in agricultural soil and sediments of neighboring water bodies such as irrigation canals could thus contribute to determine the potential risk of PTEs accumulation in the long term and the associated effects to the environment and local population.
In Bolivia, the fast-growing population along with migration of rural inhabitants to urban areas has led rapid expansion of the peri-urban zones of its main cities (Blanes 2006; Balderrama 2011). Cochabamba, the third largest city of the country, received immigrants from neighboring rural communities that led to the expansion of the peri-urban zone towards the south of the city (Mazurek 2007; Balderrama 2011; Mehta et al. 2014). Natural ecosystems and agricultural areas were urbanized, and the remaining agricultural fields are now interspersed with domestic areas and industrial activities such as automobile repair workshops, car-washing facilities, metal smelters, glass and plastic factories, and an oil refinery (Pareja et al. 2011). Due to deficient implementation of territorial planning and deficient wastes management (Mamani and Ampuero 2001; Reading et al. 2011; Cossio et al. 2021), domestic and industrial wastes may cause contamination of irrigation water, accumulation of PTEs in sediments of irrigation canals, and ultimately contaminate agricultural soils. Indeed, water contamination with PTES including manganese, zinc, copper, lead, and cadmium has been reported in certain areas of the city (Ghielmi et al. 2008, D’Abzac et al. 2020). The contamination was associated to liquid discharges from industrial activities and the dumping of solid wastes in open areas, roadsides, water canals and rivers (Ghielmi et al. 2008; Mehta et al. 2014; D’Abzac et al. 2020; Quillaguamán et al. 2021). Evaluation of PTEs concentrations in the remaining agricultural soil and sediments of irrigation canals in the peri-urban zone of the city is essential to determine whether human activity has led to their accumulation, and to assess their potential effect on the environment and human health. The objective of this study was to evaluate the concentrations of manganese (Mn), zinc (Zn), copper (Cu), lead (Pb) and cadmium (Cd) in agricultural soils and sediment of irrigation canals of two agricultural areas located in the peri-urban zone of Cochabamba, Bolivia.
2 Materials and methods
2.1 Study area
The study was conducted in Martillo and Tamborada agricultural areas (99 and 43 ha, respectively; Figure 1), located at the intersection of Districts 8 and 9 of Cochabamba city where agricultural activities encompass between 3 and 20% of the local population (Antequera 2008). The weather is characterized by a summer rainy season (November-March) and a winter dry season (April-October) with mean annual temperature of 17.5 °C, and an average year precipitation of 558 mm (Navarro and Maldonado 2002). Both agricultural areas are located in the plain zone of the valley formed by fluvio-lacustrine deposits (Renner and Velasco 2000) with soils classified as Entisols with loam and clay textures (Rocha 1998).
Maiz (Zea mayz L.) and alfalfa (Medicago sativa L.) produced in both agricultural areas supply neighboring dairy farms (Romero 2005; Herbas et al. 2017). The typical rotation includes one or two cultivation cycles of maize followed by five cycles of alfalfa cultivation after which fields are left in fallow for one year. Both areas are irrigated with water from Tamborada river, transported through unlined canals of the man-made irrigation system “Sistema Nacional de Riego N°1” (national irrigation system N°1; Gaceta Nacional de Bolivia 1948, 1945). Tamborada river receives wastewaters from neighboring urban areas and industries, but solid wastes are also dumped in the riverbed or irrigation canals (Mamani and Ampuero 2001). Small-scale automobile repair workshops and car-washing facilities encompass approximately 90% of industrial activities in the vicinity of Tamborada and Martillo. Additionally, metal smelters, galvanizing plastic and glass factories are also present in the area. Canals that irrigate Tamborada agricultural area also receive treated wastewaters from the neighboring Gualberto Villarroel oil refinery.

Figure 1: Satellite image of the study area located in the south of Cochabamba Municipality. Red points and yellow squares indicate the location of sampling points for agricultural soils and sediments, respectively, in Martillo (A) and Tamborada (B).
A grid was stablished in both agricultural areas with a minimum distance of 50 m between points using QGIS (ver. 3.22). Fourty-six points (18 at Tamborada and 28 at Martillo) were randomly selected and determined as soil sampling points. Similarly, 16 sediment sampling points were randomly determined along the irrigation canals (7 at Tamborada and 9 at Martillo), with a minimum distance of 100 m between points. One soil and sediment sample was collected at each sampling point using a soil auger (20 cm depth) at the beginning of the dry season (April 2022) and two to three weeks after the last irrigation event.
2.2 Sample analyses
Samples were air-dried at room temperature, homogenized, sieved (2 mm mesh) and stored. Soil pH and electrical conductivity (EC) were determined in a 1:2.5 (w/v) soil-water solution using a pH-meter (Mettler Toledo MP 220) and conductometer (OAKTON PC-700), respectively. Total soil organic matter (SOM) was determined by loss-on-ignition at 450°C for 4 h (Allen 1989).
The total concentrations of PTEs were determined on 1 g of soil digested with 3 ml of HClO4 (70%) at 200°C for 2 h. The solution was cooled to room temperature and diluted with distilled water to 25 ml and centrifuged (1764 g, 10 min). The concentrations of total Mn, Zn, Cu, Pb and Cd in the extracts were determined through atomic absorption spectrometry (novaA 350, Analytik Jena, Germany). Reagent blanks and sample replicates were included in the readings to ensure quality of results.
2.3 Statistical analyses
In addition to standard descriptive statistics (i.e., minimum, maximum, mean and standard deviation) the medians of PTEs concentrations were calculated because elements in soils may have a log-normal distribution (Salminen and Tarvainen 1997). Boxplots and histograms were constructed to detect outliers commonly associated to anthropogenic activity (Reimann et al. 2005; Galán et al. 2008). We evaluated the differences of soil variables pH, EC, SOM and PTEs concentrations of agricultural soils and sediments between and within agricultural areas with T-Student test for normal and homoscedastic data or with Wilcoxon Mann-Whitney test for not-normal heteroscedastic data. Principal component analyses and Pearson correlation analyses were conducted to detect association between variables. Statistical analyses were conducted in R software ver. 4.2.1 (R Core Team 2022) using car (Fox et al. 2018), dplyr (Wickham et al. 2019), factoextra (Kassambara and Mundt 2019), and Hmisc (Harrell 2022) packages.
3 Results
3.1 Soil characteristics and PTEs
The descriptive statistics for soil characteristics and PTEs for each agricultural area are presented in Table 1. The soil pH in the study area ranged from 6.8 to 8.6 with a mean value of 7.7±0.4 and without significant differences within and between agricultural areas. Electrical conductivity ranged between 95.8 and 845.0 mS cm-1 with a mean value of 291.8±162.9 mS cm-1, and with higher values for agricultural soil samples and sediment samples in Tamborada compared to those of Martillo. Soil organic matter ranged from 29.05 to 206.88 g kg-1 with a mean value of 58.61±33.16 g kg-1. In both agricultural areas, agricultural soil samples had significantly lower SOM compared to sediments samples.
Table 1. Mean ± standard deviation of soil characteristics pH, EC, SOM and potentially toxic elements Mn, Zn, Pb and Cu from agricultural soils and irrigation canals in Martillo and Tamborada. Different uppercase letters indicate significant differences between agricultural soils and sediments within each agricultural area, and different lowercase letters indicate significant differences of agricultural soils and sediments between agricultural areas (T-test or Mannp-value<0.01). Calculation of means and standard deviations of Cd was not possible due the low number of samples in which it was detected.
Martillo | Tamborada | |||
---|---|---|---|---|
Agricultural soils | Sediments | Agricultural soils | Sediments | |
pH | 7.77±0.44 Aa | 7.59±0.28 Aa | 7.75±0.48 Aa | 7.35±0.25 Aa |
EC (mS cm-1) | 209.81±83.64 Aa | 256.33±56.58 Aa | 366.16±208.61 Ab | 474.14±149.14 Ab |
SOM (g kg-1) | 45.86±11.26 Aa | 65.70±30.15 Ba | 50.15±13.91 Aa | 122.20±55.56 Bb |
Mn (mg kg-1) | 562.66±124.60 Aa | 684.81±144.87 Ba | 508.64±107.96 Aa | 451.67±182.86 Ab |
Zn (mg kg-1) | 64.23±15.06 Aa | 89.05±26.47 Ba | 82.80±9.77 Ab | 260.08±126.87 Bb |
Pb (mg kg-1) | 29.12±7.11 Aa | 34.94±8.52 Aa | 36.96±5.63 Ab | 41.98±8.98 Aa |
Cu (mg kg-1) | 33.65±7.40 Aa | 35.75±4.23 Aa | 27.45±7.63 Ab | 37.11±11.97 Ba |
According to the overall median concentration the order of PTEs was Mn>Zn>Pb>Cu with 550.75, 75.95, 32.35, and 31.95 mg of Mn, Zn, Pb and Cu per kg of soil, respectively (Figure 2). Histogram frequency distributions of Mn, Cu and Pb were not skewed while the histogram frequency distribution of Zn was positively skewed. The median and average Cd concentrations were not calculated, and the histogram frequency distribution was not plotted because this element was detected only in two agricultural soils samples (0.26 and 0.01 mg Cd kg-1) and one sediment sample (0.35 mg Cd kg-1) at Martillo. Comparisons within agricultural areas indicate that in Martillo sediment samples had significantly higher Mn and Zn concentrations compared to agricultural soil samples (Table 1). In Tamborada, sediment samples had significantly higher Zn and Cu concentrations compared to agricultural soil samples. Comparisons between agricultural areas indicate that soils samples of Tamborada had significantly higher concentrations of Zn and Pb but lower Cu concentrations compared to those of Martillo. Sediments of Tamborada had significantly higher Zn concentrations, but lower Mn concentrations compared to sediments of Martillo. No differences of Mn concentration were detected between agricultural soils of Tamborada and Martillo, and similarly, no differences of Pb and Cu concentrations were detected between sediment samples of Tamborada and Martillo
3.2 Association of soil variables and PTEs
The principal component analyses showed multivariate discrimination of sediments from Tamborada in the left quadrants of the principal component axis, while the estimated data points of agricultural soils and sediments of Martillo and agricultural soils of Tamborada were overlapped along both principal components (Figure 3). Examination of the variable loadings indicates that estimated data points for Tamborada were associated to higher Zn, SOM, Pb, and EC values while estimated data points for Martillo were associated to higher values of Cu, Mn and soil pH. Accordingly, correlation analyses indicated statistically significant positive associations of Zn, Pb and Cu while Mn and Zn were negatively associated (Table 2).
Table 2. Correlation matrix of potentially toxic elements Mn, Zn, Pb, Cu. Below the diagonal are the Pearson correlation values (asterisks indicate values statistically different from cero), and above the diagonal are the levels of significance (p-value). Cadmium was not included in the plots due to low number of samples in which it was detected.
Mn | Zn | Pb | Cu | |
---|---|---|---|---|
Mn | - | 0.01 | 0.26 | 0.45 |
Zn | -0.34* | - | <0.01 | <0.01 |
Pb | -0.14 | 0.62* | - | 0.02 |
Cu | -0.10 | 0.39* | 0.29* | - |
4 Discussion
4.1 Soil characteristics and PTEs accumulation
Soils in the study area are neutral to alkaline, and EC indicates saline soil conditions likely attributed to calcium and carbonates accumulation (Gutiérrez and Cáceres 2018). Higher EC in Tamborada indicates higher soil salinity compared to Martillo, which might be associated to differences in parent material (Ordovician quartzitic sandstone are interspersed with outcrops of Silurian marine sediments with diverse clasts in the area; Renner and Velasco 2000), but differences in salts transported by irrigation water may also contribute to this difference (Machado and Serralheiro 2017). The higher SOM values in sediments samples compared to agricultural soils in Martillo and Tamborada can be attributed to organic matter accumulation in irrigation canals due to slower organic matter decomposition caused by anoxic conditions during wet periods (Bot and Benites 2005), and to the potential negative effect of accumulated PTEs (see below) on microbial processes such as respiration (Sauvé et al. 1997; Oorts et al. 2006).
Inspection of Mn, Cu and Pb histogram frequency distributions does not indicate significant deviations from Gaussian distribution, suggesting the absence of anomalous high values commonly attributed to anthropogenic activity (Salminen and Tarvainen 1997; Reimann and Garrett 2005). On the contrary, the positively skewed histogram of Zn indicated anthropogenic contribution to the concentration of this element in the study area. Given that PTEs are naturally present in soils and also common contaminants in zones with intense human activity (Davies 1983), the concentrations observed are likely the result of the naturally occurring background levels and the effect of domestic and industrial activities. The median concentrations reported may thus be considered approximations to Mn, Zn, Pb and Cu baseline concentrations for the area.
The average concentrations of Mn, Pb, Cu of agricultural soils and sediments of Martillo and Tamborada were within the natural variation of uncontaminated soils (40-900 mg Mn, 2-200 mg Pb and 2-50 mg Cu, 0.01-2.60 mg Cd kg-1; Swaine 1956; István and Jones 1997; Barceloux 1999; Oorts 2013; Smolders and Mertens 2013; Steinnes 2013; Uren 2013). Accordingly, Pb concentrations did not surpass the maximum permissible values for agricultural soils established by the Bolivian legislation (100 mg Pb kg-1; Estado Plurinacional de Bolivia 2015), and Cu concentrations did not surpass European and North American thresholds above which soils are considered moderately contaminated (>100 mg Cu kg-1; Parlamento Europeo 1984; Sauvé et al. 1997; Ministry of the Environment Finland 2007), while Mn threshold values have not been established due to its low potential to exert toxic effects on the environment and human health (Barceloux 1999; Uren 2013; Bošković-Rakočević et al. 2014).
Average Zn concentration of agricultural soils in both agricultural areas and in sediment samples of Martillo were also within natural variation of uncontaminated soils (10-100 mg Zn kg-1; Mertens and Smolders 2013) but sediments of Tamborada surpassed this range (260.1±126.9 mg Zn kg-1). Similarly, these extreme values surpassed the maximum permissible value established by the Bolivian legislation (200 mg Zn kg-1; Estado Plurinacional de Bolivia 2015), indicating contamination of irrigation canals with this element. These extreme values were also observed in the boxplot of Tamborada (Figure 2) and were discriminated in the left quadrant of the principal component analyses (Figure 3).
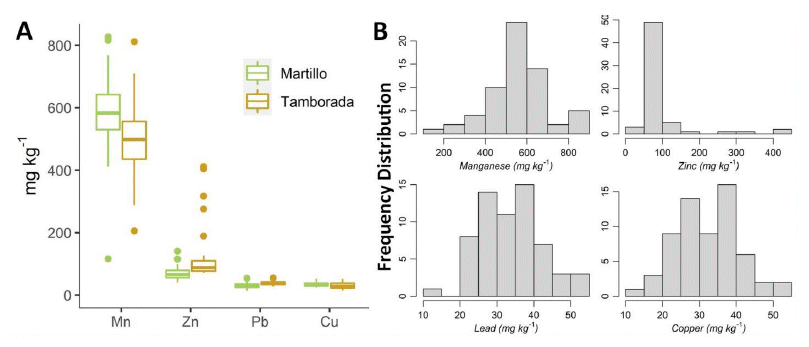
Figure 2: Boxplots (A) and histograms (B) of potentially toxic elements Mn, Zn, Pb and Cu. Boxes show the 25-75% interquartile range, whiskers (25 and 75 quartiles ± 1.5* interquartile range) and outliers. Cadmium was not included in the plots due to low number of samples in which it was detected.
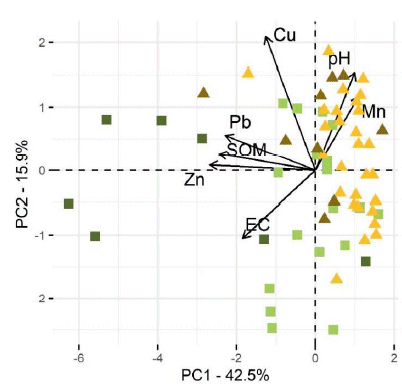
Figure 3: Multivariate principal component analyses conducted on the soil properties pH and electrical conductivity (EC), soil organic matter (SOM) and potentially toxic elements manganese (Mn), zinc (Zn), lead (Pb), and cooper (Cu) in Martillo (▲) and Tamborada (■) peri-urban agricultural areas. Light yellow and brown triangles indicate agricultural soils and sediments from Martillo, respectively. Light green and dark green squares indicate agricultural soils and sediments from Tamborada, respectively.
Significantly higher Mn and Zn concentrations in sediment compared to soil samples in Martillo, and significantly higher Zn and Cu concentrations in sediments compared to soil samples of Tamborada suggest accumulation of these elements in irrigation canals. Similarly, the higher Zn and Pb concentrations observed in agricultural soils, and higher Zn concentration in sediments of Tamborada compared to those of Martillo suggest their accumulation in this agricultural area. These results along with the multivariate discrimination and variable loadings of PCA suggest that the agricultural areas would be exposed to different pollution loads. It is possible that Tamborada would be exposed to higher Zn and Pb loads, while Martillo would be exposed to higher Cu and Mn loads.
Accumulation of Zn, Pb and Cu in agricultural fields and irrigation canals of Tamborada could be associated to the use of contaminated wastewaters from the neighboring Gualberto Villarroel oil refinery. The positive associations of Zn, Pb and Cu also suggest a common contamination source. Indeed, Pb- and Zn-compounds (e.g., tetra-ethyl lead, zinc dialkyldithiophosphate) are used as anti-knock agents for gasoline production pollution (Cassells and Dodds 1946; Barnes et al. 2001; Steinnes 2013), and Cu is used as catalyst for octane boosters and antiknock additives production (e.g., diphenyl carbonate; Takeuchi 2012), which may be present in wastewater of the oil refinery. Inspection of Pb, Zn and Cu spatial variation (supplementary material S1 and S2) indicates that higher concentrations of these elements are located in the entrance of vehicles and farm tractors or near roads with high automobile traffic in both agricultural areas. Atmospheric depositions from tyre dust and debris along with the spillage of automobile oils would thus be important Zn, Pb and Cu sources (Onianwa et al. 2001; Kreider et al. 2010; Kibblewhite 2018). Additionally, wastes from automobile repair and washing facilities, metal smelters, plastics and rubber production factories in the area may also have contributed to Zn and Pb accumulation (Fleming and Parle 1977; Mertens and Smolders 2013; Steinnes 2013), while plumbing corrosion may have contributed to Cu accumulation (Oorts 2013).
Manganese and Zn accumulation in irrigation canals of Martillo may be linked to the presence of a glass factory and smelt and galvanizing industries close to this agricultural area (Barceloux 1999). Indeed, glass-refining and coloring chemicals such as zinc oxide and manganese oxide may be present in wastes of the glass industry (Varun et al. 2012) and Zn alloys are commonly present in effluents from galvanizing activities (Mertens and Smolders 2013; Mahurpawar 2015). The negative association between Zn and Mn (Table 2), however, suggest different pollution loads or contamination sources of these elements. Determination of element sources is crucial to manage PTEs accumulation in agricultural soil of this peri-urban area. Moreover, given that the long-term use of wastewaters for irrigation may lead to soil contamination and increase environmental and health risk (Mapanda et al. 2005), monitoring of water quality is crucial to determine the PTEs pollution loads and accumulation rates.
The detection of Cd in three samples in Martillo area suggest that the geochemical Cd concentration might be below the detection limit of our study (0.14 mg l-1; detection and quantification limits are presented in supplementary material S3), and that the detected concentrations may be considered anomalous values. Focal Cd accumulation could be attributed to solid wastes (e.g. batteries) that are dumped in the vicinity of agricultural fields or in irrigation canals (Mamani and Ampuero 2001). Accordingly, inspection of spatial distribution of these samples indicates that focal accumulation points were located at the entrance of the irrigation canal and close to a high traffic road, supporting this explanation. Nevertheless, Cd concentrations did not surpass the maximum permissible value for agricultural soils stablished by the Bolivian legislation (2 mg Cd kg-1; Estado Plurinacional de Bolivia 2015). Evaluations with lower detection and quantification limits are required to accurately determine Cd background values and accumulation patterns.
4.2 Potential bioavailability and hazard
Despite that total element concentrations are commonly used to determine soil contamination; they do not reflect the proportion of PTEs that are available to living organisms (Hettiarachchi and Pierzynski 2004). The interactions of PTEs with soil characteristics determine their bioavailability instead. For example, under the alkaline and saline soil conditions, complexation and precipitation with carbonates phosphates, sulphates or hydroxyles (Oorts 2013; Steinnes 2013; Rengel 2015), and fixation on reactive sites of minerals, oxides (Buekers et al. 2008; Mertens and Smolders 2013) or organic matter (Steinnes 2013) may have taken place and reduced their bioavailability. Natural attenuation overtime (i.e., aging) through diffusion of PTEs into micro-pores, occlusion into organic matter and sorption on clay particles (Sauvé et al. 1997; Steinnes 2013; Wijayawardena et al. 2015) along with pH increase during waterlogged periods of irrigation canals could further reduce availability of PTEs (Alloway 2003; Smolders and Mertens 2013). However, rhizosphere acidification caused by root exudates (commonly observed in alkaline environments as a strategy of plants to increase nutrients availability), may take place and counter the chemical effect of soil characteristics on PTEs availability (Alloway 2003; Smolders and Mertens 2013). We acknowledge the need to assess the bioavailability of PTEs under the specific soil conditions of the study area to determine their transfer rate to plants. However, potential hazard to human health associated to Mn accumulation is unlikely due to its low bioaccumulation in plants (Barceloux 1999; O’Neal and Zheng 2015). In fact, health risks have been linked to chronic exposure to high Mn concentration only (e.g., dust or fumes with >1000 mg kg-1; Barceloux 1999; Lucchini et al. 2012). Similarly, transfer of accumulated Zn and Cu to the trophic chain and potential hazard to human health is unlikely due to homeostatic maintenance in normal ranges and their phytotoxic effects at high concentrations (Cai et al. 2009; Alloway 2013; Oorts 2013). Nevertheless, accumulated Mn, Zn and Cu may be toxic to soil microorganisms, annelids, nematodes, arthropods, microorganisms and affect soil processes such as organic matter decomposition (Sauvé et al. 1997; Oorts et al. 2006). The extent of their effect depends on species sensitivity (Blackwell et al. 1998; Mertens and Smolders 2013; Oorts 2013; La Torre et al. 2018), and on the presence of attenuating factors such as plant species that reduce their concentrations in the soil (La Torre et al. 2018; Reeves et al. 2018). Given that ecotoxicity thresholds for these elements are highly variable and overlap with natural background concentrations (Broos et al. 2007; Mertens and Smolders 2013; Oorts 2013), the potential toxic effect to terrestrial organisms associated to their accumulation cannot be inferred from their total concentrations alone.
On the contrary to essential elements, Pb and Cd do not have a biological role and their accumulation in agricultural soils may pose a risk to the environment and human health. Given that the current concentrations are within the range of uncontaminated soils, these elements may not affect crops quality in the studied areas. In fact, transfer of Pb to plants is generally small except in cases of high Pb concentrations (Albering et al. 1999; Miller et al. 2004; Steinnes 2013), and transfer of Cd has been reported to increase above total soil concentrations of 2 mg kg-1 (Fukushima et al. 1973; de Vries et al. 2007). In addition to the soil characteristics that could promote their stabilization (alkaline saline soils), factors such as metabolic regulation of their absorption (Bi et al. 2010), and competition in the channels of cell membranes with other accumulated elements (e.g., Mn, Zn and Cu) would also contribute to reduce their transfer to plants (Cai et al. 2009; Alloway 2013). We acknowledge, however, that the determination of Pb and Cd bioconcentration and bioaccumulation factors (used to assess human exposure through ingestion of vegetables and meet; de Vries et al., 2007), as well as determination of the critical metal concentrations (a threshold above which crops quality could be affected; de Vries et al., 2007) is essential to determine the risk associated to their accumulation, particularly in irrigation canals. Given that the accumulation of these elements was likely related to the use of wastewaters from an oil refinery and to the dumping of wastes in the vicinity of agricultural areas, improvement of solid and liquid waste management facilities is essential to manage their accumulation, particularly because their accumulation could lead to soil contamination in the long-term (Mapanda et al. 2005).
5 Conclusions
Intense human activity in the southern peri-urban zone of Cochabamba caused Zn and Cu accumulation in irrigation canals, as well as Pb and Zn accumulation in agricultural soils of Tamborada, likely associated to the use of wastewaters from a neighboring oil refinery and automobile-related pollution. Manganese and Zn accumulated in irrigation canals of Martillo, likely associated to industrial activities (i.e., glass factory, metal smelters) in the area, while focal accumulation of Cd could be attributed to solid wastes dumped in the vicinity of agricultural fields. Concentrations of PTEs were within natural range variation of uncontaminated soils, except for Zn concentrations in irrigation canals of Tamborada which surpassed the maximum permissible values established by the Bolivian legislation. The improvement of solid and liquid waste management facilities is essential to manage PTEs accumulation in agricultural soils. Moreover, determination of contamination sources and monitoring of water quality is crucial to determine the rate of PTEs accumulation and the risk of contamination in the long-term. The median Mn, Zn, Pb and Cu concentrations reported (550.74, 75.95, 32.35 and 31.95 mg kg-1, respectively) are suggested as approximations to the baseline concentrations of these elements for the area, while detailed studies are required to determine the baseline value and accumulation patterns of Cd.